Discovery of quantum spin liquid puts physics in a spin
Quantum computing and artificial intelligence may be the stuff of science fiction, but they could be closer now than ever before thanks to the discovery of quantum spin liquid
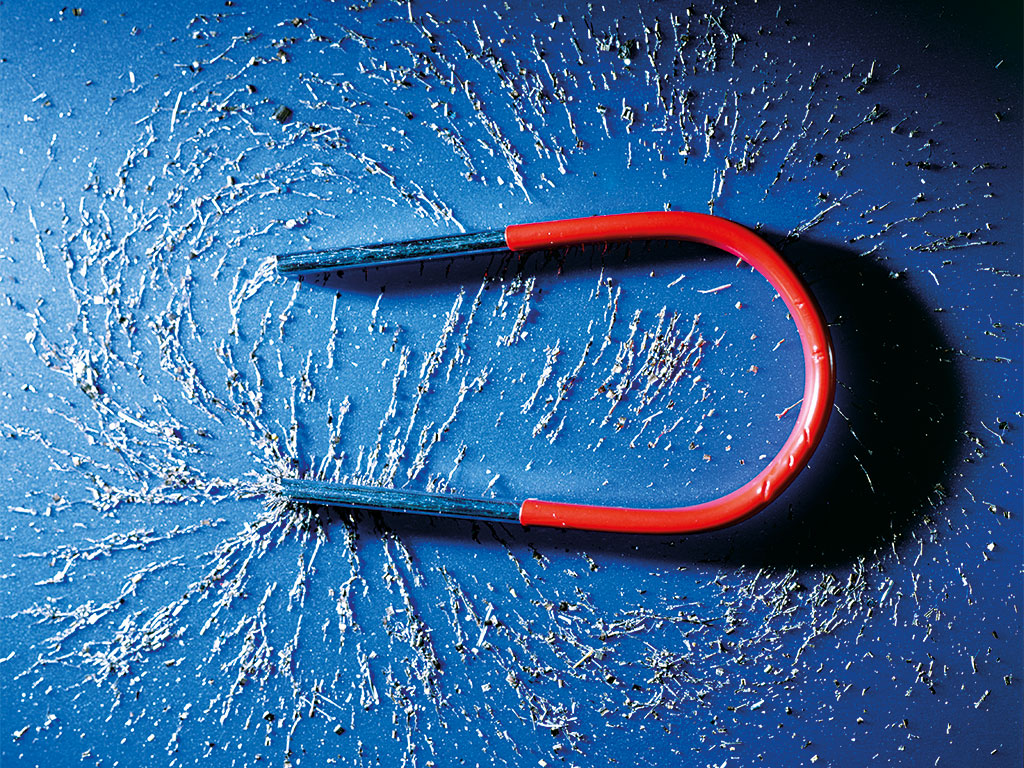
The discovery of quantum spin liquid as a standalone state of matter has ignited the physics world
April was an exciting month for physics, and for quantum physics in particular. Along with the three states of matter that we all know and interact with constantly – liquid, gas and solid – we now have another to explore: quantum spin liquid (QSL). The breakthrough, which was first revealed in the journal Nature Materials, signifies a very important step in the field, one that could potentially hold the key to a new world in the not-too-distant future.
The discovery of QSL has been years in the making; it was first proposed by physicist Philip Anderson over 40 years ago and was then developed further by theoretical physicist Alexei Kitaev in 2006, when he found an ingenious way of making the theory work in practice. In the subsequent decade, an impressive array of talents and skills were required to make the next steps. “Overcoming the challenges in this experiment involved putting together a team of people with complementary skills: chemistry, experimental physics, and theoretical physics”, said Dr Arnab Banerjee, a physicist at the US Department of Energy’s Oak Ridge National Laboratory.
Spinning around
In order to understand what quantum spin liquid is, it is necessary to have an understanding of materials and, specifically, magnetic materials. All materials are comprised of atoms, and their movement determines the material’s state. In liquids, atoms are free to move around, but do so together in patterns. In gases, they move around without correlation. In solids, atoms may shake but do not move around, and have greater correlation. The factor that determines the state of matter is, of course, temperature. However, there is also another factor – the quantum concept of ‘zero-point motion’, which refers to the incessant motion of atoms, regardless of temperature.
The atoms of magnetic materials differ in that they act like tiny magnets, a phenomenon that occurs as a result of their ‘spins’. “Magnetic materials can be described theoretically by a lattice of electron spins. These spins can be thought of as elementary bar magnets”, said Dr Johannes Knolle, one of the QSL paper’s co-authors at Cambridge University’s Cavendish Laboratory. When temperatures are lowered, motion comes to a stop and spins are arranged in regular patterns. Interestingly, “in quantum spin liquids, spins remain disordered down to very low temperatures”, Knolle said. “In these states, exotic properties are expected to emerge: although the spins do not form a pattern, there is a quantum entanglement between spins.”
The discovery of QSL has been years in the making; it was first proposed by physicist Philip Anderson over 40 years ago
Banerjee elaborated further: “Under special circumstances in a material, the tiny spins interact with each other pretty strongly but, paradoxically, even at absolute zero temperature they cannot decide how to align. This indecisiveness is called ‘quantum frustration’. The disorder is further enhanced in small spins by their zero-point motion. We call this state a ‘quantum spin liquid’. Here, the spins are random, but they still interact strongly enough to move together to create waves and excitations.”
Critical breakthrough
“First we had to identify a material where there was a good reason to believe that a QSL might be present, and then we had the difficult job of purifying this material and getting a correct sample”, Banerjee said. Once alpha-ruthenium trichloride was chosen as the magnetic material to investigate, ensuring the sample’s integrity became the next big challenge, with the stoichiometry alone taking over a year to complete.
When the sample was ready, the team used neutrons to impart a small amount of energy into the spins that, when hit, were excited. “The neutrons, on the other hand, lose the energy and momentum which they have given to the spins. We can detect these exiting neutrons and figure out how much energy and momentum they have lost, and this is how we can tell what the spins are actually doing”, said Banerjee.
This method of ‘neutron scattering’ enables scientists to measure a signal for the aforementioned excitations, which they call a ‘response function’. The response function measured during the experiment did not correspond to what is found in ordinary materials, which are either ferromagnetic, meaning the spin orientation is in one direction only, or antiferromagnetic, where the spin is disordered. Instead, the response function was similar to what was calculated for the state predicted more than 10 years ago by Kitaev – the Kitaev QSL.
Implication station
“What is remarkable is that the energy band we saw is very unique – arising from fractionalised quasiparticles called Majorana fermions. Fractionalisation is the tendency of quantum systems to behave differently to the sum of their parts. It is the queer property that makes fundamental particles like electrons, which are naively indivisible, seem to break up into smaller fractions of themselves – i.e. quasiparticles. In our case, the Majorana fermion is such a quasiparticle”, Banerjee explained. “Our experiment also proves that fractionalisation can be directly measured and physically understood in a real solid-state material if the right approach is used. This may be significant from an application standpoint, since it gives access to properties of matter not accessible otherwise.”
First presented by Kitaev, it is the special properties of Majorana fermion excitations that can finally create a thermally stable quantum analogue of the binary digit – the qubit. “If these quasiparticles can be harnessed and controlled, they can pave the way for qubits, which will have the special property that they are topologically protected from decoherence, most notably temperature and thermal fluctuations.” This step would make quantum computation practically viable by solving the biggest current challenge – quantum decoherence and the resultant loss of information.
While a very important step has been made, Banerjee is wary and notes that it is still not quite Kitaev QSL. “It is a new state of matter, because magnetism in these materials has topological properties, which normal matter does not have”, he said. “What we found is an energy band, which resembles the expectation from a material that is in very close proximity to the QSL. We still have to do research to get to the true QSL. But knowing that we are close is a big achievement.”
“Our work is only a first small step in this direction”, Knolle said. “For example, one has to understand the properties of these spin liquids in more detail. We are yet to learn how to manipulate Majorana fermions in these materials in a controlled fashion.”
Unsurprisingly, the physicists’ interpretation of this discovery is practical, logical and measured. That does not, however, make its implications any less exciting. The paper published in Nature Materials is indeed a small step towards Kitaev QSL, but it is a step nevertheless, and one that heralds a world of possibilities: quantum computing, calculations that are still impossible at present, AI, and, ultimately, a cosmos of technology and potential that can usher in a new era for humankind.